Revealing Pulmonary Structure and Function with Hyperpolarized Magnetic Resonance Imaging
Magnetic resonance imaging (MRI) is a common tool used to study the structure and function of bodily tissues in health and disease. Although MRI has undergone decades of technological advances and improvements, it still is fundamentally limited by low signal sensitivity.1,2 The technique of hyperpolarization may be one important approach to overcoming this limitation by temporarily enhancing the magnetization of polarizable atomic nuclei. The enhanced hyperpolarized (HP) state improves the MRI signal sensitivity more than 10,000-fold in most chemical species.1,2 Moreover, because the improved sensitivity results from altered nuclear spin orientations rather than ionizing radioactivity, HP MRI is safe for repeated use in humans.
One HP technique is based on increasing the nuclei spin polarization of gases, such as helium-3 (3He) or xenon-129 (129Xe). The long-time agent of choice for HP gas MRI has been 3He because it is both highly polarizable and completely chemically inert. In recent years, the field of MRI has been shifting toward the use of 129Xe because of its lower cost and wider availability. An important application of HP gas MRI is to study the structure and physiology of the pulmonary system.3,4 MRI of HP-gas-inflated lungs clearly distinguishes between the low-signal lung tissue and the high-signal air sac. This differentiation elucidates lung architecture and airway gas exchange.5 With easy uptake into the bloodstream and tissue, 129Xe is also being used for monitoring blood flow and receptor-targeted imaging, facilitating brain and kidney imaging, and broadening the applications of HP MRI technology.4–6
Another, more recent, HP strategy uses injectable carbon-13 (13C)‑based biological substrates. As with HP gas, this liquid-state HP MRI allows real-time dynamic imaging of substances that, although present in the body, are usually found in concentrations too low to detect using conventional proton-based MRI. The detection of individual molecular signatures informs about the localization of molecular binding sites and biochemical transformations in cells. Insight into the physiology of tissues (e.g., lung, liver, and heart) is gained by quantifying downstream metabolic products of the 13C-containing substrate (i.e., lactate). This approach is also being used to investigate processes that underlie the pathogenesis of many diseases by probing metabolism, tissue perfusion, and pH.7–9
As medical diagnostics and treatments evolve toward personalized models, the ability to measure metabolic processes regionally and in real-time is becoming increasingly important. Localized assessment of cellular activity can highlight disease-related changes that may not yet be reflected by conventional imaging of overall tissue structure or function.10–12 Follow-up HP MRI sessions may also assist in assessing treatment effectiveness.
The unique combination of safety and sensitivity makes HP MRI a powerful tool. The principal shortcoming of HP MRI is that the enhanced signal lasts from only a few seconds to a few minutes at most. This short half-life of nuclear magnetic polarization necessitates the creation of polarized agents that must take place on the premises. Also necessary is the development of special imaging techniques to enhance signal detectability.
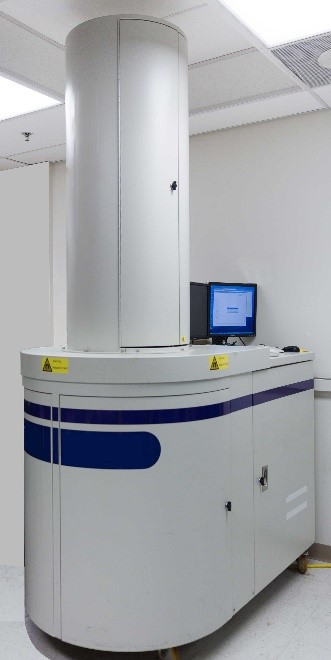
The National Institutes of Health’s (NIH’s) Office of Research Infrastructure Programs (ORIP) Shared Instrumentation Program (S10) contributes to the advancement of biomedical research by providing access to state-of-the-art instrumentation that otherwise would not be available to many scientists. In the last 5 years (Fiscal Years [FY] 2013–2017), the S10 program awarded more than 500 instruments in a broad range of technologies, including nine hyperpolarizers. The Functional and Metabolic Imaging Group (FMIG) in the University of Pennsylvania, led by Dr. Rahim Rizi, Professor of Radiology, has conducted research on the non-invasive assessment of lung structure, function, and metabolism for the last 22 years. During FY 2009–2014 with S10 funding, Dr. Rizi acquired five instruments to support FMIG’s research; these instruments include polarizers for gas and 13C MRI for functional and metabolomic studies.
The Hypersense™ instrument uses the Dynamic Nuclear Polarization (DNP) technique to hyperpolarize 13C and produce imaging tracers. These tracers are used in small animal-based experiments to study pulmonary perfusion and inflammation, and metabolism of various cancers. A newer instrument, SPINlab™, produces sterile hyperpolarized 13C samples at volumes five times larger and at a 20-fold throughput increase compared to Hypersense™. Tracers synthesized with SPINlab™ support large animal- and human-based studies, including implementation of diagnostic protocols for different types of cancer and for the evaluation of cancer treatments. HP gas MRI at the FMIG is supported by the Xemed™ XeBox-E10 instrument. XeBox produces HP 129Xe at a rate of about 6 liters per hour, enabling the quantitative assessment of human lung function and structure. The ability to measure regional lung ventilation opens possibilities for early detection of pathological conditions and their diagnoses. Small animal experiments are supported by a 9.4T MRI scanner and a microCT imager.
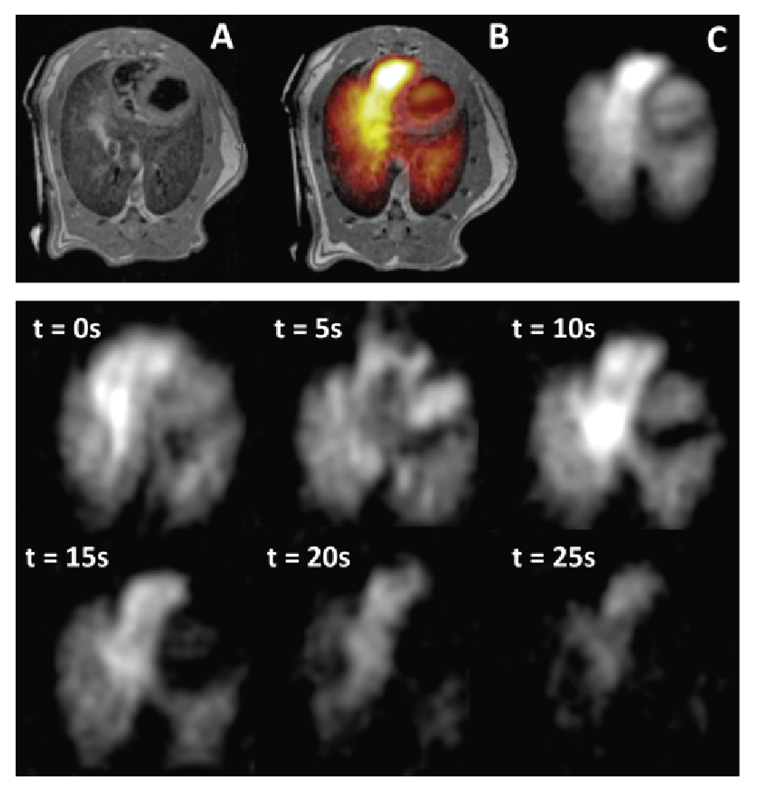
According to Dr. Rizi, “With its dramatically improved temporal resolution, HP MRI enables the direct in vivo observation of dynamic biological processes, including the changes in cellular metabolism characteristic of essentially all disease processes.” Recent work by the FMIG has demonstrated that HP gas can detect smoking-related lung injuries more accurately than conventional diagnostic methods, such as pulmonary function tests.11 In another NIH-funded preclinical study, the FMIG is using gas-based HP MRI to quantify lung function and predict the side effects of radiation therapy in lung cancer.
The FMIG uses HP 13C MRI to investigate the physiology of ventilator-induced lung injury, with the goal of developing improved ventilator protocols to reduce the widespread inflammation that often precedes injury progression. The laboratory also aims to improve the assessment of lungs viable for transplantation and to better predict transplant rejection. To quantify the rejection process and to potentially identify factors that can slow it, the FMIG studies the changes in the lactate-to-pyruvate ratio, a marker of lung injury and disease. The FMIG also employs HP 13C MRI in animal models to examine a wide range of disorders, including prostate and lung cancers, cardiac disease and heart failure, renal failure, liver conditions, and several neural pathologies, aiming to translate the imaging protocols to human applications.13–19
In summary, the FMIG with its unique collection of instruments and special scientific expertise serves as a local and regional shared resource, supporting the development and uses of HP MRI techniques. Instruments described here also have been successfully used in studies of cardiac metabolism, kidney recovery after ischemia, heart and liver and lung redox state, animal models of liver cancer, and altered energy metabolism in diabetes.
For more information, visit on ORIP’s S10 program page.
References
1Golman K, Olsson LE, Axelsson O, Mansson S, Karlsson M, Petersson JS. Molecular imaging using hyperpolarized 13C. The British Journal of Radiology. 2003;76:S118–27.
2Ardenkjaer-Larsen JH, Fridlund B, Gram A, Hansson G, Hansson L, Lerche MH, et al. Increase in signal-to-noise ratio of > 10,000 times in liquid-state NMR. Proceedings of the National Academy of Sciences. 2003;100(18):10158–63.
3Kadlecek SJ, Emami K, Fischer MC, Ishii M, Yu J, Woodburn JM, et al. Imaging physiological parameters with hyperpolarized gas MRI. Progress in Nuclear Magnetic Resonance Spectroscopy. 2005;47(3–4):187–212.
4Ruppert K. Biomedical imaging with hyperpolarized noble gases. Reports on Progress in Physics. 2014;77(11):116701.
5Rao M, Stewart NJ, Norquay G, Griffiths PD, Wild JM. High resolution spectroscopy and chemical shift imaging of hyperpolarized (129)Xe dissolved in the human brain in vivo at 1.5 tesla. Magnetic Resonance in Medicine. 2016;75(6):2227–34.
6Driehuys B, Cofer GP, Pollaro J, Mackel JB, Hedlund LW, Allan Johnson G. Imaging alveolar-capillary gas transfer using hyperpolarized 129Xe MRI. Proceedings of the National Academy of Sciences. 2006;103(48):18278–83.
7Shaghaghi H, Kadlecek S, Siddiqui S, Pourfathi M, Hamedani H, Clapp J, et al. Ascorbic acid prolongs the viability and stability of isolated perfused lungs: A mechanistic study using (31)P and hyperpolarized (13)C nuclear magnetic resonance. Free Radical Biology and Medicine. 2015;89:62–71.
8Shaghaghi H, Kadlecek S, Deshpande C, Siddiqui S, Martinez D, Pourfathi M, et al. Metabolic spectroscopy of inflammation in a bleomycin-induced lung injury model using hyperpolarized 1-13C pyruvate. NMR in Biomedicine. 2014;27(8):939–47.
9Pourfathi M, Xin Y, Kadlecek SJ, Cereda MF, Profka H, Hamedani H, et al. In vivo imaging of the progression of acute lung injury using hyperpolarized [1-13 C] pyruvate. Magnetic Resonance in Medicine. 2017;78(6):2106–15.
10Mills GH, Wild JM, Eberle B, Van Beek EJR. Functional magnetic resonance imaging of the lung. British Journal of Anaesthesia. 2003;91(1):16–30.
11Hamedani H, Kadlecek SJ, Ishii M, Xin Y, Emami K, Han B, et al. Alterations of regional alveolar oxygen tension in asymptomatic current smokers: Assessment with hyperpolarized (3)He MR imaging. Radiology. 2015;274(2):585–596.
12Mugler JP, Altes TA. Hyperpolarized (129)Xe MRI of the human lung. Journal of Magnetic Resonance Imaging. 2013;37(2):313–31.
13Kurhanewicz J, Vigneron DB, Brindle K, Chekmenev EY, Comment A, Cunningham CH, et al. Analysis of cancer metabolism by imaging hyperpolarized nuclei: Prospects for translation to clinical research. Neoplasia. 2011;13(2):81–97.
14Nelson SJ, Kurhanewics J, Vigneron DB, Larson PEZ, Harzstark AL, Ferrone M, et al. Metabolic imaging of patients with prostate cancer using hyperpolarized [1-13C] pyruvate. Science Translational Medicine. 2013;5(198):198ra108.
15Gallagher FA, Kettunen MI, Brindle KM. Biomedical applications of hyperpolarized 13C magnetic resonance imaging. Progress in Nuclear Magnetic Resonance Spectroscopy. 2009;55(4):285–95.
16Park I, Larson PEZ, Gordon JW, Carvajal L, Chen HY, Bok R, et al. Development of methods and feasibility of using hyperpolarized carbon‐13 imaging data for evaluating brain metabolism in patient studies. Magnetic Resonance in Medicine. 2018;doi: 10.1002/mrm.27077 [Epub ahead of print].
17Laustsen C, Østergaard JA, Lauritzen MH, Nørregaard R, Bowen S, Søgaard LV, et al. Assessment of early diabetic renal changes with hyperpolarized [1- (13)C] pyruvate. Diabetes Metabolism Research Reviews. 2013;29(2):125–9.
18Cunningham CH, Lau JY, Chen AP, Geraghty BJ, Perks WJ, Roifman I, et al. Hyperpolarized 13C metabolic MRI of the human heart: Initial experience. Circulation Research. 2016;119(11):1177–82.
19DeVience SJ, Lu X, Proctor J, Rangghran P, Melhem ER, Gullapalli R, et al. Metabolic imaging of energy metabolism in traumatic brain injury using hyperpolarized [1-13C] pyruvate. Scientific Reports. 2017;7(1):1907.